No hay productos en el carrito
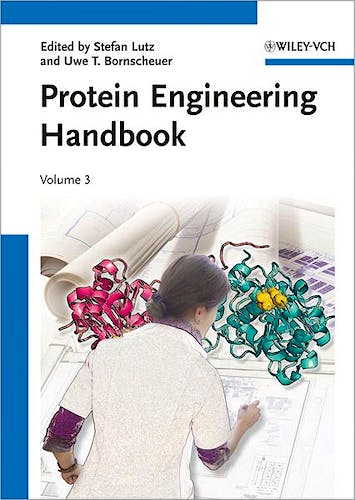
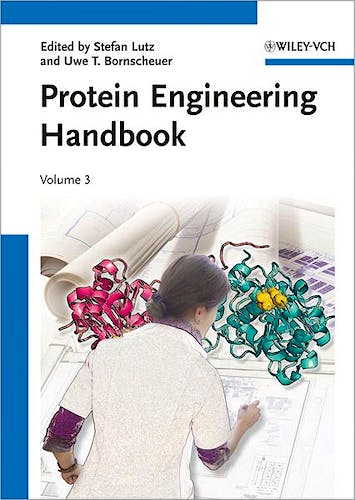
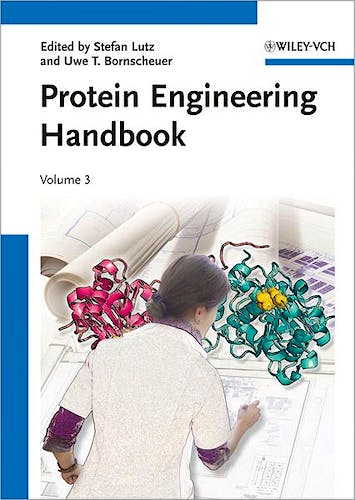
Protein Engineering Handbook, Vol. 3
Lutz, S. — Bornscheuer, U.
1ª Edición Octubre 2012
Inglés
Tapa dura
502 pags
1055 gr
18 x 24 x 3 cm
ISBN 9783527331239
Editorial WILEY
LIBRO IMPRESO
-5%
224,67 €213,44 €IVA incluido
216,03 €205,23 €IVA no incluido
Recíbelo en un plazo de
2 - 3 semanas
LIBRO ELECTRÓNICO
-5%
200,79 €190,75 €IVA incluido
193,07 €183,41 €IVA no incluido
Acceso On Line
Inmediato
Description
This introduction collects 17 innovative approaches to engineer novel and improved proteins for diverse applications in biotechnology, chemistry, bioanalytics and medicine. As such, key developments covered in this reference and handbook include de novo enzyme design, cofactor design and metalloenzymes, extremophile proteins, and chemically resistant proteins for industrial processes. The editors integrate academic innovations and industrial applications so as to arrive at a balanced view of this multi-faceted topic.
Throughout, the content is chosen to complement and extend the previously published
two-volume handbook by the same editors, resulting
in a superb overview of this burgeoning field.
Table of Contents
Preface
List of Contributors
1 Dirigent Effects in Biocatalysis 1
Bettina M. Nestl, Bernd A. Nebel, and Bernhard Hauer
- 1.1 Introduction 1
- 1.2 Dirigent Proteins 3
- 1.3 Solvents and Unconventional Reaction Media 4
- 1.3.1 Ionic Liquids 7
- 1.3.2 Microemulsions and Reversed Micelles Systems 10
- 1.4 Structure and Folding 12
- 1.5 Structured and Unstructured Domains 14
- 1.6 Isozymes, Moonlighting Proteins, and Promiscuity: Supertalented Enzymes 19
- 1.7 Conclusions 22
- Acknowledgment 23
- References 23
2 Protein Engineering Guided by Natural Diversity 29
James T. Kratzer, Megan F. Cole, and Eric A. Gaucher
- 2.1 Approaches 29
- 2.1.1 Ancestral Sequence Reconstruction (ASR) 30
- 2.1.2 Ancestral Mutation Method 31
- 2.1.3 Reconstructing Evolutionary Adaptive Paths (REAP) 32
- 2.2 Protocols 34
- 2.2.1 Practical Steps to Using ASR 34
- 2.2.2 Reconstructing Evolutionary Adaptive Paths: A Focused Application of ASR 36
- 2.3 Future Directions 38
- 2.3.1 Industrial Applications 40
- 2.3.2 Biomedical 41
- 2.3.3 Drug Discovery 41
- 2.3.4 Paleobiology 42
- 2.3.5 Synthetic Biology 43
- 2.3.6 Experimental Validation of ASR 43
- 2.4 Conclusions 44
- References 44
3 Protein Engineering Using Eukaryotic Expression Systems 47
Martina Geier and Anton Glieder
- 3.1 Introduction 47
- 3.2 Eukaryotic Expression Systems 48
- 3.2.1 Yeast Expression Platforms 48
- 3.2.1.1 Saccharomyces cerevisiae 48
- 3.2.1.2 Pichia pastoris 51
- 3.2.1.3 Pichia angusta 54
- 3.2.1.4 Alternative Yeasts 55
- 3.2.2 Filamentous Fungi 56
- 3.2.3 Insect Cells 58
- 3.2.4 Mammalian Cell Cultures 59
- 3.2.5 Transgenic Animals and Plants 61
- 3.2.6 Cell-Free Expression Systems 61
- 3.3 Conclusions 63
- References 65
4 Protein Engineering in Microdroplets 73
Yolanda Schaerli, Balint Kintses, and Florian Hollfelder
- 4.1 Introduction 73
- 4.2 Droplet Formats 75
- 4.2.1 “Bulk” Emulsions 75
- 4.2.1.1 Catalytic Selections Involving DNA Substrates 76
- 4.2.1.2 Using the Droplet Compartment to Form a Permanent Genotype-Phenotype Linkage for Selections of Binders 77
- 4.2.2 Double “Bulk” Emulsions 78
- 4.2.3 Microfl uidic Droplets 79
- 4.3 Perspectives 83
- Acknowledgments 84
- References 84
5 Folding and Dynamics of Engineered Proteins 89
Michelle E. McCully and Valerie Daggett
- 5.1 Introduction 89
- 5.2 Proof-of-Principle Protein Designs 90
- 5.2.1 FSD-1, a Heterogeneous Native State and Complicated Folding Pathway 91
- 5.2.2 a3D, a Dynamic Core Leads to Fast Folding and Thermal Stability 94
- 5.2.3 Three-Helix Bundle Thermostabilized Proteins 96
- 5.2.4 Top7, a Novel Fold Topology 97
- 5.2.5 Other Rosetta Designs 100
- 5.3 Proteins Designed for Function 102
- 5.3.1 Ligands 103
- 5.3.1.1 Metal-Binding Four-Helix Bundles, the Effectiveness of Negative Design 103
- 5.3.1.2 Peptide Binding 105
- 5.3.2 Enzymes 106
- 5.3.2.1 Retro-Aldol Enzyme, Accommodating a Two-Step Reaction 106
- 5.3.2.2 Kemp Elimination Enzyme, Rigid Active Site Geometry Promotes Catalysis 108
- 5.4 Conclusions and Outlook 110
- Acknowledgments 111
- References 112
6 Engineering Protein Stability 115
Ciarán Ó’Fágáin
- 6.1 Introduction 115
- 6.2 Power and Scope of Protein Engineering to Enhance Stability 116
- 6.2.1 Thermal Stabilizations 116
- 6.2.1.1 Potential Therapeutics: Rational Design with Computational Support 116
- 6.2.1.2 Analytical Tools: Green Fluorescent Protein and Luciferase 128
- 6.2.1.3 “Stiffening” a Protein by Gly-to-Pro Replacement: Methyl Parathion Hydrolase 128
- 6.2.2 Thermal Is Not the Only Stability: Oxidative and Other Chemical Stabilities 129
- 6.2.2.1 Oxidative Stability 129
- 6.2.2.2 Stabilization against Aldehydes and Solvents 130
- 6.2.2.3 Alkaline Tolerance 131
- 6.3 Measurement of a Protein’s Kinetic Stability 132
- 6.3.1 Materials and General Hints 132
- 6.3.2 Thermal Stability 132
- 6.3.2.1 Thermal Profi le 132
- 6.3.2.2 Thermal Inactivation 133
- 6.3.3 Measurement of Oxidative Stability 134
- 6.3.4 Stability Analysis and Accelerated Degradation Testing 135
- 6.3.4.1 Set-Up 136
- 6.3.4.2 Analysis of Results 137
- 6.4 Developments in Protein Stabilization 137
- References 139
7 Enzymes from Thermophilic Organisms 145
Tamotsu Kanai and Haruyuki Atomi
- 7.1 Introduction 145
- 7.2 Hyperthermophiles 146
- 7.3 Enzymes from Thermophiles and Their Reactions 146
- 7.4 Production of Proteins from (Hyper)Thermophiles 148
- 7.5 Protein Engineering of Thermophilic Proteins 154
- 7.6 Cell Engineering in Hyperthermophiles 156
- 7.7 Future Perspectives 157
- References 157
8 Enzyme Engineering by Cofactor Redesign 163
Malgorzata M. Kopacz, Frank. Hollmann, and Marco W. Fraaije
- 8.1 Introduction 163
- 8.2 Natural Cofactors: Types, Occurrence, and Chemistry 164
- 8.3 Inorganic Cofactors 165
- 8.4 Organic Cofactors 168
- 8.5 Redox Cofactors 169
- 8.5.1 Nicotinamide Cofactor Engineering 170
- 8.5.2 Heme Cofactor Engineering 173
- 8.5.2.1 Reconstitution of Myoglobin 174
- 8.5.2.2 Artificial Metalloproteins Based on Serum Albumins 175
- 8.5.3 Flavin Cofactor Engineering 176
- 8.6 Concluding Remarks 180
- References 181
9 Biocatalyst Identifi cation by Anaerobic High-Throughput Screening of Enzyme
Libraries and Anaerobic Microorganisms 193
Helen S. Toogood and Nigel S. Scrutton
- 9.1 Introduction 193
- 9.2 Oxygen-Sensitive Biocatalysts 194
- 9.2.1 Flavoproteins 194
- 9.2.2 Iron-Sulfur-Containing Proteins 195
- 9.2.3 Other Causes of Oxygen Sensitivity 197
- 9.3 Biocatalytic Potential of Oxygen-Sensitive Enzymes and Microorganisms 198
- 9.3.1 Old Yellow Enzymes (OYEs) 198
- 9.3.2 Enoate Reductases 200
- 9.3.3 Other Enzymes 202
- 9.3.4 Whole-Cell Anaerobic Fermentations 202
- 9.4 Anaerobic High-Throughput Screening 203
- 9.4.1 Semi-Anaerobic Screening Protocols 204
- 9.4.2 Anaerobic Robotic High-Throughput Screening 205
- 9.4.2.1 Purifi ed Enzyme versus Whole-Cell Extracts 207
- 9.4.2.2 Indirect Kinetic Screening versus Direct Product Determination 208
- 9.4.3 Potential Extensions of Robotic Anaerobic High-Throughput Screening 209
- 9.5 Conclusions and Outlook 210
- References 210
10 Organometallic Chemistry in Protein Scaffolds 215
Yvonne M. Wilson, Marc Dürrenberger, and Thomas R. Ward
- 10.1 Introduction 215
- 10.1.1 Concept 215
- 10.1.2 Considerations for Designing an Artifi cial Metalloenzyme 216
- 10.1.2.1 Organometallic Complex 216
- 10.1.2.2 Biomolecular Scaffold 218
- 10.1.2.3 Anchoring Strategy 219
- 10.1.2.4 Advantages and Disadvantages of the Different Anchoring Modes 221
- 10.1.2.5 Spacer 222
- 10.1.3 Other Key Developments in the Field 223
- 10.1.4 Why Develop Artifi cial Metalloenzymes? 223
- 10.2 Protocol/Practical Considerations 226
- 10.2.1 Protein Scaffold 226
- 10.2.1.1 Determination of Free Binding Sites 226
- 10.2.2 Organometallic Catalyst 228
- 10.2.2.1 Synthesis of [Cp*Ir(biot-p-L)Cl] 229
- 10.2.2.2 N'-(4-Biotinamidophenylsulfonyl)-Ethylenediamine TFA Salt 230
- 10.2.3 Combination of Biotinylated Metal Catalyst and Streptavidin Host 231
- 10.2.3.1 Binding Affi nity of the Biotinylated Complex to Streptavidin 231
- 10.2.4 Catalysis 232
- 10.2.4.1 Catalysis Controls 232
- 10.3 Goals 234
- 10.3.1 Rate Acceleration 234
- 10.3.2 High-Throughput Screening 234
- 10.3.2.1 Considerations for Screening of Artificial Metalloenzymes 235
- 10.3.3 Expansion of Substrate Scope 236
- 10.3.4 Upscaling 236
- 10.3.5 Potential Applications 237
- 10.4 Summary 237
- Acknowledgments 237
- References 238
11 Engineering Protease Specificity 243
Philip N. Bryan
- 11.1 Introduction 243
- 11.1.1 Overview 243
- 11.1.2 Some Basic Points 244
- 11.1.2.1 Mechanism for a Serine Protease 244
- 11.1.2.2 Measuring Specifi city 244
- 11.1.2.3 Binding Interactions 245
- 11.1.3 Nature versus Researcher 247
- 11.1.3.1 P1 Specifi city of Chymotrypsin-like Proteases 247
- 11.1.3.2 The S1 Site of Subtilisin 247
- 11.1.3.3 The S4 Site of Subtilisin 250
- 11.1.3.4 Other Subsites in Subtilisin 250
- 11.1.3.5 Kinetic Coupling and Specifi city 251
- 11.2 Protocol and Practical Considerations 251
- 11.2.1 Remove and Regenerate 251
- 11.2.2 Engineering Highly Stable and Independently Folding Subtilisins 252
- 11.2.3 Engineering of P4 Pocket to Increase Substrate Specifi city 253
- 11.2.4 Destroying the Active Site in Order to Save It 254
- 11.2.5 Identifying a Cognate Sequence for Anion-Triggered Proteases Using the Subtilisin Prodomain 255
- 11.2.6 Tunable Chemistry and Specifi city 257
- 11.2.7 Purification Proteases Based on Prodomain–Subtilisin Interactions and Triggered Catalysis 258
- 11.2.8 Design of a Mechanism-Based Selection System 259
- 11.2.8.1 Step 1: Ternary Complex Formation 259
- 11.2.8.2 Step 2: Acylation 263
- 11.2.8.3 Steps 3 and 4: Deacylation and Product Release 265
- 11.2.9 Evolving Protease Specifi city Regulated with Anion Cofactors by Phage Display 266
- 11.2.9.1 Construction and Testing of Subtilisin Phage 266
- 11.2.9.2 Random Mutagenesis and Transformation 267
- 11.2.9.3 Selection of Anions 267
- 11.2.9.4 Evolving the Anion Site 267
- 11.2.9.5 Catch-and-Release Phage Display 267
- 11.2.9.6 Conclusions 269
- 11.2.10 Evolving New Specifi cities at P4 269
- 11.3 Concepts, Challenges, and Visions on Future Developments 270
- 11.3.1 Design Challenges 270
- 11.3.2 Challenges in Directed Evolution 271
- 11.3.2.1 One Must Go Deep into Sequence Space 271
- 11.3.2.2 Methods Which Maximize Substrate Binding Affinity Are Not Productive 272
- 11.3.2.3 The Desired Protease May Be Toxic to Cells 272
- 11.3.3 The Quest for Restriction Proteases 272
- 11.3.3.1 Not All Substrate Sequences Are Created Equal 273
- 11.3.4 Final Thoughts: Gilded or Golden? 273
- Acknowledgments 274
- References 274
12 Polymerase Engineering: From PCR and Sequencing to Synthetic Biology 279
Vitor B. Pinheiro, Jennifer L. Ong, and Philipp Holliger
- 12.1 Introduction 279
- 12.2 PCR 281
- 12.3 Sequencing 281
- 12.3.1 First-Generation Sequencing 282
- 12.3.2 Next-Generation Sequencing Technologies 284
- 12.4 Polymerase Engineering Strategies 288
- 12.5 Synthetic Informational Polymers 291
- References 295
13 Engineering Glycosyltransferases 303
John McArthur and Gavin J. Williams
- 13.1 Introduction to Glycosyltransferases 303
- 13.2 Glycosyltransferase Sequence, Structure, and Mechanism 304
- 13.3 Examples of Glycosyltransferase Engineering 307
- 13.3.1 Chimeragenesis and Rational Design 307
- 13.3.2 Directed Evolution 310
- 13.3.2.1 Fluorescence-Based Screening 311
- 13.3.2.2 Reverse Glycosylation Reactions 312
- 13.3.2.3 ELISA-Based Screens 313
- 13.3.2.4 pH Indicator Assays 314
- 13.3.2.5 Chemical Complementation 314
- 13.3.2.6 Low-Throughput Assays 314
- 13.4 Practical Considerations for Screening Glycosyltransferases 315
- 13.4.1 Enzyme Expression and Choice of Expression Vector 315
- 13.4.2 Provision of Acceptor and NDP-donor Substrate 315
- 13.4.3 General Considerations for Microplate-Based Screens 317
- 13.4.4 Promiscuity, Profi ciency, and Specifi city 317
- 13.5 Future Directions and Outlook 318
- References 319
14 Protein Engineering of Cytochrome P450 Monooxygenases 327
Katja Koschorreck, Clemens J. von Bühler, Sebastian Schulz, and Vlada B.
Urlacher
- 14.1 Cytochrome P450 Monooxygenases 327
- 14.1.1 Introduction 327
- 14.1.2 Catalytic Cycle of Cytochrome P450 Monooxygenases 328
- 14.1.3 Redox Partner Proteins 329
- 14.2 Engineering of P450 Monooxygenases 330
- 14.2.1 Molecular Background for P450 Engineering 330
- 14.2.2 Altering Substrate Selectivity and Improving Enzyme Activity 332
- 14.2.2.1 Rational and Semi-Rational Design 332
- 14.2.2.2 Directed Evolution and Its Combination with Computational Design 336
- 14.2.2.3 Decoy Molecules 338
- 14.2.3 Improving Solvent and Temperature Stability of P450 Monooxygenases 340
- 14.2.3.1 Solvent Stability 341
- 14.2.3.2 Thermostability 342
- 14.2.4 Improving Recombinant Expression and Solubility of P450 Monooxygenases 343
- 14.2.4.1 N-Terminal Modifi cations 344
- 14.2.4.2 Modifi cations within the F-G Loop 346
- 14.2.4.3 Improving Expression by Rational Protein Design and Directed Evolution 348
- 14.2.5 Engineering the Electron Transport Chain and Cofactors of P450s 349
- 14.2.5.1 Genetic Fusion of Proteins 349
- 14.2.5.2 Enzymatic Fusion and Self-Assembling Oligomers 352
- 14.3 Conclusions 354
- References 355
15 Progress and Challenges in Computational Protein Design 363
Yih-En Andrew Ban, Daniela Röthlisberger-Grabs, Eric A. Althoff, and Alexandre
Zanghellini
- 15.1 Introduction 363
- 15.2 The Technique of Computational Protein Design 363
- 15.2.1 Principles of Protein Design 363
- 15.2.2 A Brief Review of Force-Fields for CPD 364
- 15.2.3 Optimization Algorithms for Fixed-Backbone Protein Design (P1') 368
- 15.3 Protein Core Redesign, Structural Alterations, and Thermostabilization 371
- 15.3.1 Protein Core Redesign and de novo Fold Design 371
- 15.3.2 Computational Alteration of Protein Folds 373
- 15.3.2.1 Loop Grafting 374
- 15.3.2.2 de novo Loop Design 375
- 15.3.2.3 Fold Switching 376
- 15.3.2.4 Fold Alteration: Looking Ahead 377
- 15.3.2.5 Computational Optimization of the Thermostability of Proteins 377
- 15.4 Computational Enzyme Design 380
- 15.4.1 de novo Enzyme Design 380
- 15.4.1.1 Initial Proofs-of-Concept 380
- 15.4.1.2 Review of Recent Developments 382
- 15.4.2 Computational Redesign of the Substrate Specifi city of Enzymes 383
- 15.4.2.1 Fixed-Backbone and Flexible-Backbone Substrate Specifi city Switches 383
- 15.4.2.2 Limitations and Feedback Obtained from Experimental Optimization Attempts 385
- 15.4.3 Frontiers in Computational Enzyme Design 386
- 15.5 Computational Protein–Protein Interface Design 388
- 15.5.1 Natural Protein–Protein Interfaces Redesign 389
- 15.5.2 Two-Sided de novo Design of Protein Interfaces 390
- 15.5.3 One-Sided de novo Design of Protein Interfaces 392
- 15.5.4 Frontiers in Protein–Protein Interaction Design 393
- 15.6 Computational Redesign of DNA Binding and Specifi city 394
- 15.7 Conclusions 396
- References 396
16 Simulation of Enzymes in Organic Solvents 407
Tobias Kulschewski and Jürgen Pleiss
- 16.1 Enzymes in Organic Solvents 407
- 16.2 Molecular Dynamics Simulations of Proteins and Solvents 408
- 16.3 The Role of the Solvent 410
- 16.4 Simulation of Protein Structure and Flexibility 411
- 16.5 Simulation of Catalytic Activity and Enantioselectivity 413
- 16.6 Simulation of Solvent-Induced Conformational Transitions 414
- 16.7 Challenges 415
- 16.8 The Future of Biocatalyst Design 416
- References 417
17 Engineering of Protein Tunnels: The Keyhole–Lock–Key Model for
Catalysis by Enzymes with Buried Active Sites 421
Zbynek Prokop, Artur Gora, Jan Brezovsky, Radka Chaloupkova, Veronika Stepankova,
and Jiri Damborsky
- 17.1 Traditional Models of Enzymatic Catalysis 421
- 17.2 Defi nition of the Keyhole–Lock–Key Model 422
- 17.3 Robustness and Applicability of the Keyhole–Lock–Key Model 424
- 17.3.1 Enzymes with One Tunnel Connecting a Buried Active Site to the Protein Surface 424
- 17.3.2 Enzymes with More than One Tunnel Connecting a Buried Active Site to the Protein Surface 433
- 17.3.3 Enzymes with One Tunnel Between Two Distinct Active Sites 436
- 17.4 Evolutionary and Functional Implications of the Keyhole–Lock–Key Model 437
- 17.5 Engineering Implications of the Keyhole–Lock–Key Model 438
- 17.5.1 Engineering Activity 442
- 17.5.2 Engineering Specifi city 443
- 17.5.3 Engineering Stereoselectivity 443
- 17.5.4 Engineering Stability 443
- 17.6 Software Tools for the Rational Engineering of Keyholes 444
- 17.6.1 Analysis of Tunnels in a Single Protein Structure 445
- 17.6.2 Analysis of Tunnels in the Ensemble of Protein Structures 445
- 17.6.3 Analysis of Tunnels in the Ensemble of Protein–Ligand Complexes 447
- 17.7 Case Studies with Haloalkane Dehalogenases 448
- 17.8 Conclusions 450
- References 452
Index 465
Author Information
Stefan Lutz holds a B. S. degree from the Zurich University of Applied Sciences (Switzerland), and a M.S. degree from the University of Teesside (UK). He then obtained a Ph.D. from the University of Florida and spent three years as a Postdoc with Stephen Benkovic at Pennsylvania State University under a fellowship of the Swiss National Science Foundation. Since 2002 he has been a Chemistry professor at Emory University in Atlanta, Georgia (USA). The research in the Lutz laboratory focuses on the structure-function relationship of proteins through combinatorial protein engineering and design.
Uwe Bornscheuer studied Chemistry at the University of Hannover (Germany), where he obtained a Ph. D. at the Institute of Technical Chemistry. He then spent a postdoctoral year at the University of Nagoya, Japan, before returning to Germany to join the Institute of Technical Biochemistry at the University of Stuttgart. Since 1999 he has been Professor for Biotechnology and Enzyme Catalysis at the University of Greifswald. His main research interest is the application of engineered enzymes in the synthesis of optically active compounds and in lipid modification.
© 2025 Axón Librería S.L.
2.149.0